What’s Cooking With CRISPR?
Simmering Advances in Genome Editing at NIH
BY MICHAEL TABASKO, THE NIH CATALYST
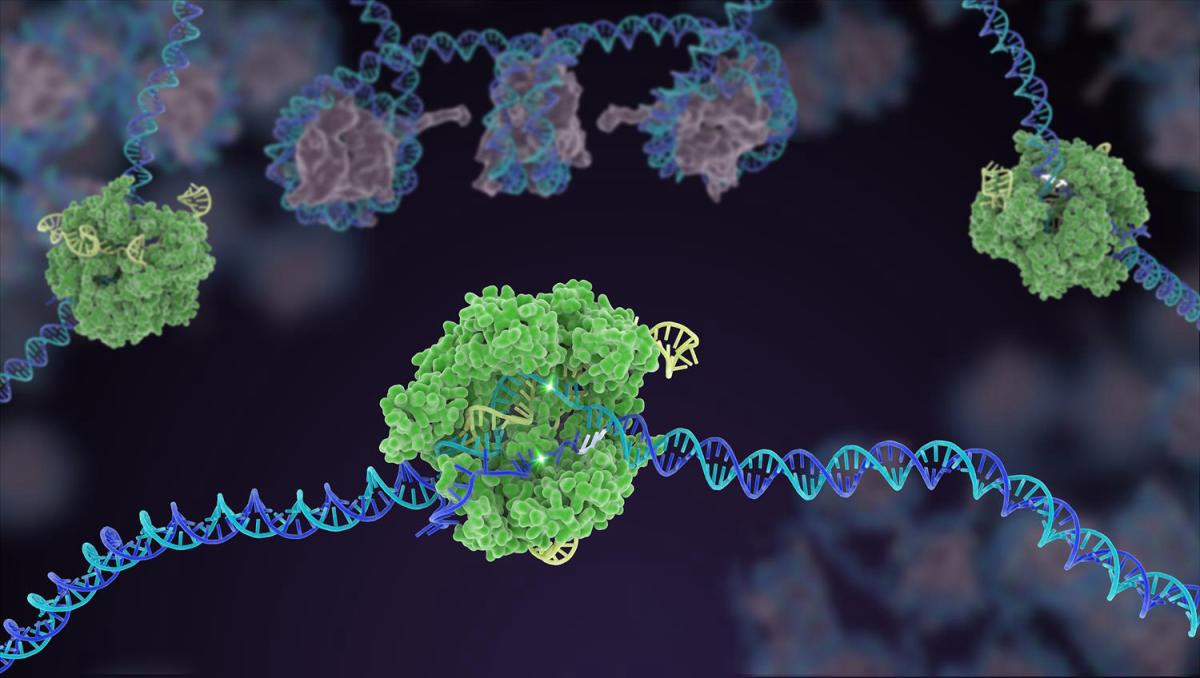
CREDIT: JANET IWASA
In the gene-editing tool CRISPR, a small strand of guide RNA identifies a specific sequence of DNA. Then, enzymes such as Cas9 (green) cuts the double-stranded DNA (blue and purple) in two places, removing the specific chunk. Scientists can then use different approaches to modify the gene and repair the DNA break. Caption adapted from the NIH Gene Editing Digital Media Kit.
It’s been over a decade since CRISPR-based gene editing technology carried the ingredients of great promise into the biomedical kitchen. The technique is remarkably accurate and easy to use compared with prior gene-editing methods, and so the scientific community got to work refining it as a precision medicine tool. Myriad applications for CRISPR ensued, and the blue-sky goal seemed straightforward: One day, we would be able to simply snip disease from our genome.
That day hasn't arrived for all diseases and all people, but we are alluringly close. Consider Victoria Gray, a patient with sickle cell disease (SCD) who received the first FDA-approved gene therapy at Sarah Cannon Cancer Institute at TriStar Health (Nashville, Tennessee) using a version of CRISPR called Cas9 to eliminate her symptoms. So far, so good. But significant obstacles in bringing CRISPR to the masses remain. The high cost and tight regulations contribute to the slow development of gene-editing therapies, according to a Government Accountability Office report.
In this issue of the Catalyst, read about sickle cell cures and gene therapy pioneered by John Tisdale and others at the NIH.
Nevertheless, CRISPR technology has proven curative in an exceptionally brief timeframe. And the NIH has been in the midst of the CRISPR revolution. Here, we detail some history and current progress.
Invented in 2012, the elegant CRISPR-Cas9 tool is adapted from a bacterial immune system in which microbes incorporate a sequence of foreign DNA into their own genetic code to recognize and defend against viral invaders. Eugene Koonin, an NIH distinguished investigator in NLM, was among the first to recognize, in 2005, that “spacer DNA” in the clustered regularly interspaced short palindromic repeats—that is, CRISPR—regions of bacteria and archaea matched sequences of bacteriophages and thus might be a part of an adaptive immune system (PMID:16545108).
In 2020, Jennifer Doudna and Emmanuelle Charpentier, who realized CRISPR's potential for human disease therapy, were awarded the Nobel Prize in Chemistry for the discovery. The "Cas9" part refers to one particularly efficient enzyme that is commonly used in the technology to make a double-stranded DNA break. A short sequence of guide RNA shepherds the cutting enzyme into a cell to dock at a programmed location in the genome. Researchers can then insert or delete genetic material and alter the function of a target gene using a variety of approaches.
Listen to Jennifer Doudna deliver the WALS NIH Director’s Lecture: The Future of CRISPR: What’s Ahead for Genome Editing.
At first, monogenic inherited disorders such as SCD were considered the most logical diseases to target with CRISPR techniques. But a steady rollout of research using the technology to treat diseases beyond SCD, from blindness (PMID: 38709228) to in vivo treatment of protein-folding disorders (PMID: 34215024), is expanding CRISPR’s recipe book to benefit more people. Complementing that work are several CRISPR-based advances being pursued at NIH.
Editing out infectious and inherited diseases
Attacking the hepatitis B virus (HBV) from different angles is illustrative of how gene editing might be used to combat infectious diseases. Although an HBV vaccine exists, there is no curative treatment for those already infected.
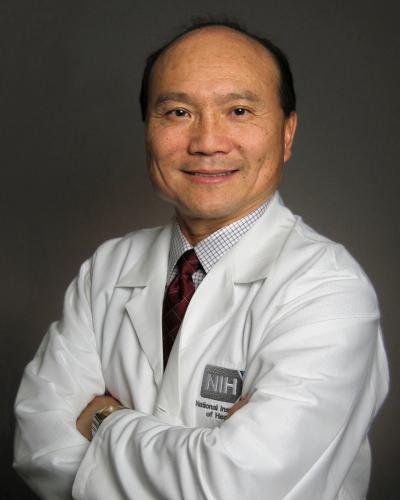
CREDIT: NIDDK
Jake Liang
“Like many people, we are using CRISPR-Cas technology or a derivative of it as a tool to edit genes of interest,” said Jake Liang, an NIH distinguished investigator at NIDDK’s Liver Diseases Branch. His group edited a human stem cell line to express a known HBV-resistant gene in hepatocytes and showed that those cells indeed became resistant to HBV infection (PMID: 34853804). “This [result] is proof of concept that we can use CRISPR editing to confer immunity to certain infections,” said Liang.
Another angle is to alter a virus’s genome and render it harmless. For example, epigenetic mechanisms could be used to disrupt the genome of HBV as it hides within infected liver cells (PMID: 38015841). That strategy could represent one therapeutic approach, and Liang notes that CRISPR can make such epigenetic changes by turning genes on or off without permanently changing the genome, thus reducing the risk of unintended mutations. Others have harnessed in vivo delivery of CRISPR to show that cleaving the viral genome is feasible in a mouse model of chronic HBV (PMID: 33473359).
In vivo delivery is an area of substantial progress. One way to envelop and deliver CRISPR in the body is by packaging it in lipid nanoparticles, or LNPs. Compared with commonly used viral vectors for reprogramming cellular behavior, LNPs are easier to manufacture and have more cargo capacity to deliver bulkier molecular payloads.
What’s more, the fat-derived molecules naturally gravitate to the liver when injected intravenously. In their quest for an elusive hepatitis C vaccine, the Liang lab is testing how LNPs might be used as a vehicle to deliver potential hepatitis C immunogens directly to the liver.
Infectious diseases such as herpes, HIV, and human papillomavirus could also land on CRISPR’s chopping block by using epigenetic or gene-editing approaches, and possibly LNP delivery methods. “I see a real future for this technology for any number of these viruses that stay in the system for years and decades,” said Liang.
According to Liang, gene therapy efforts to treat liver diseases have been ongoing for decades, and CRISPR technology has the potential to cure a whole slew of hereditary liver conditions with lethal complications such as hemochromatosis, alpha-1-antitrypsin deficiency, and other congenital pediatric liver diseases. “Like sickle cell disease, they are all well defined. We know the mutation and can target that,” he said.
Also targetable are inborn errors of immunity (IEI), a group of inherited conditions that disrupt the immune system and can result in severe or life-threatening infections, excessive inflammation, or immune dysregulation. Although gene therapy has been used to the benefit of some of those patients, there is room for improvement. Suk See De Ravin, senior research physician and chief of NIAID’s Gene Therapy Development Unit, is developing new gene therapy and cell therapy approaches to treat IEI that are safer and more robust.
In one approach, De Ravin’s team used CRISPR to insert a functional gene to correct a form of IEI in a mouse model and in human cells. They used new techniques to enhance the DNA repair pathway and better manage the cellular response to DNA damage and were able to achieve over 60% genetic correction, resulting in significantly improved immune cell function (PMID: 34086870).
New work at De Ravin’s lab used CRISPR-Cas9 base editors to precisely correct a single-base mutation without significantly disrupting other parts of the genomic sequence (PMID: 39413163). Base editing nicks a single strand of DNA, avoiding a double-strand break that could trigger unwanted mutations or cellular responses.
According to De Ravin, those findings provided robust efficacy and safety data to support a first-in-human stem cell base editing clinical trial to repair an IEI-causing mutation to treat patients with chronic granulomatous disease. A second clinical trial was FDA approved to repair a mutation to treat patients with X-linked severe combined immunodeficiency. “Our lab is excited to extend this highly precise approach for repair of gene mutations that cause other IEIs without involving use of [viral vectors],” she said.
Optimizing vehicles and cargo
LNPs may have an affinity for the liver, but they are showing promise to deliver CRISPR-based therapy in vivo to other organs, too.
Like SCD, Fanconi anemia is an inherited bone marrow disorder and searching for a gene therapy cure is a focus of Andre Larochelle, senior investigator at NHLBI’s Laboratory of Regenerative Therapies for Inherited Blood Disorders. Fanconi anemia leads to abnormal production of oxygen-carrying red blood cells and infection-fighting white cells, putting patients at risk for infection, anemia, bleeding disorders, and cancer. Those patients’ fragile cells also have a DNA repair defect and poorly tolerate the stress of being modified outside the body in a lab.
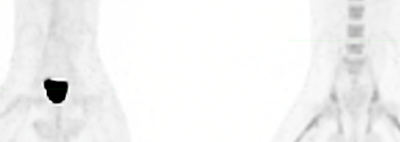
CREDIT: NORIKO SATO AND KEVIN QUIROZ CACEDA
Before (left) and after (right) positron-emission tomography-computed tomography scan images of an animal model showing marked increase in radiotracer uptake in the bone marrow after infusion with lipid nanoparticles (LNP). In the postinfusion image on the right, darker colors indicate successful LNP-mediated delivery of mRNA, providing the first evidence of LNP targeting the marrow in a large animal model. According to the Larochelle lab, the advancement is a pivotal step toward realizing in vivo gene therapy applications for the treatment of inherited hematological disorders.
In a pivotal step toward realizing in vivo gene editing for inherited bone marrow disorders in humans, preliminary findings in nonhuman primate biodistribution studies showed that LNPs infused intravenously can be taken up by the bone marrow. “While the detection of LNPs in the bone marrow is encouraging," said Larochelle, "the majority of LNPs seem to be sequestered or degraded by the innate immune system.”
To overcome those barriers, his team is conjugating LNPs with antibodies known to bind to stem cells in the bone marrow and testing methods to bypass the immune capture problem so that more LNPs successfully reach the marrow and deliver their CRISPR payload.
Once optimized, these sorts of approaches could be made available to patients around the world and be adapted to other disorders. “The same lipid nanoparticle could encapsulate a different RNA or DNA template to apply the same principle to a different disease,” said Larochelle. “The goal is to apply this process to as many diseases as possible.”
Extinguishing cancer
CRISPR is also being used as a de facto search engine to probe the inner workings of disease. Cas9 machinery can systematically knock out gene function in a mutant cell line and reveal weak spots that could then be exploited to develop therapies. This technology, known as CRISPR screens, can find genetic and epigenetic targets that explain why certain cancers are resistant to treatment, for example.
Javed Khan, a senior investigator at NCI’s Genetics Branch, leverages cutting-edge CRISPR screens to engineer reporter cell lines that track protein expression, thus uncovering vulnerabilities in aggressive pediatric cancers such as rhabdomyosarcoma and neuroblastoma. His team tests over 2,000 compounds contained in a screening platform developed by NCATS and the Molecular Targets Program, which includes FDA-approved and experimental drugs, to identify therapies that suppress the expression or activity of PAX3-FOXO1, a central cancer-driver gene specific to rhabdomyosarcoma. “Furthermore, using CRISPR gene knockout and activation technologies, we are identifying resistance or vulnerability mechanisms to develop powerful gene engineering methods to improve the efficacy of CAR T cells against these aggressive cancers,” Khan told the Catalyst. CAR refers to chimeric antigen receptors that allow T cells to recognize and destroy cancer cells.
CREDIT: NCI
Rosa Nguyen
Similarly, Rosa Nguyen, a Lasker Clinical Research Scholar at NCI’s Pediatric Oncology Branch, is working to understand why pediatric solid tumors such as neuroblastoma evade CAR T-cell therapy and uses CRISPR screens in her research. Often, the tumor environment can shut down an immune attack. Her lab found that modifying CAR T cells to express certain cytokines had potent antitumor activity in preclinical models (PMID: 36631162). That therapy is moving towards clinical trials for children with neuroblastoma. Other efforts in her lab focus on combinatorial CAR T-cell therapy, for example, with low-dose radiopharmaceutical agents that remodel the tumor environment and enhance the function of CAR T cells.
Will it even work?
“A lot of my work is to figure out relevant animal models for human disease and use those models to test whether gene knock out or correction by CRISPR would work,” said Cynthia Dunbar, NIH distinguished investigator at NHLBI’s Molecular Hematopoiesis lab. “They allow you to quickly design and carry out experiments to test if gene editing can pathophysiologically reverse the phenotype or not.”
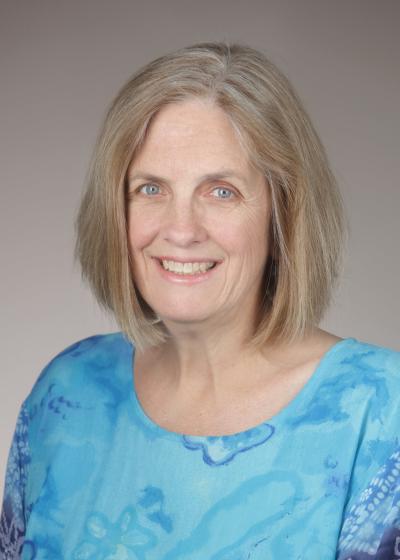
CREDIT: NHLBI
Cynthia Dunbar
Sometimes, the answer is no. For example, the rare genetic RUNX1 deficiency is another inherited bone marrow disease with an elevated risk of cancer and the RUNX1 Foundation was interested in seeing whether CRISPR gene editing could delete the mutation. Dunbar’s team used CRISPR to knock out the function of the RUNX1 gene and to reproduce the phenotype in a nonhuman primate model. Doing so allowed them to get critical insights into the different steps of marrow failure and platelet development before and after transplanting corrected cells back into the animals. Such models most closely resemble disease and therapeutic processes in humans.
But unlike SCD or cystic fibrosis, on which the gene mutation is limited to specific base pairs, RUNX1 mutations can occur all over the gene and each family is unique. “When we gave the animals the corrected cells, we found that they were outcompeted by the mutant cells,” said Dunbar (PMID: 36322931). “It was an important experiment because it showed that you’re never going to correct enough cells, and CRISPR gene editing at this point doesn’t make sense.”
Similar experiments went the other way, as with Diamond-Blackfan anemia, yet another inherited bone marrow disorder. In that case, healthy stem cells were shown to outcompete stem cells carrying the Diamond-Blackfan mutation, suggesting that if one could correct the mutation, then those edited cells might quickly dominate and result in a successful transplant.
The Dunbar lab is also using their models to test the long-term effect of edited stem cells in the body. One CRISPR approach uses a DNA repair pathway called homology-directed repair, which is known to be remarkably precise with a low chance of introducing genetic errors. But it might not work with all cell types, and Dunbar’s animal models showed that hematopoietic stem cells edited with homology-directed repair did not engraft well compared with those edited with a lentiviral approach (PMID: 38508195).
“So, it shows that we have a ways to go with [homology-directed repair],” said Dunbar, noting a wave of interest in emerging CRISPR techniques such as base editing. She stresses the importance of a rigorous process beginning with in vitro assays and moving through relevant animal models before bringing forward therapies for clinical trials. “You have to go stepwise for these totally novel approaches [in which] you don’t really know what’s going to happen.”
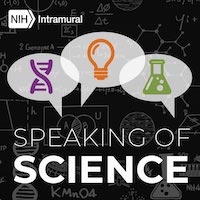
Learn more about Dunbar’s research on this episode of the “Speaking of Science” podcast.
Gene editing tech may be trending toward maturity but needs more time to cook. A “CRISPR-for-all” future will depend upon support for ongoing research, lowering costs and improving accessibility, and addressing ethical concerns. According to that same Government Accountability Office report, “Policy decisions made in the near future may affect the way in which human gene editing to treat or prevent disease is regulated, covered by health insurance providers, and accepted by society.”
If you’re a visual and auditory learner, watch and listen to this musical video explaining CRISPR-Cas9 by A Capella Science.
This page was last updated on Monday, January 6, 2025