Why Studying Bacteria Matters
I have been thinking a lot recently about how the tools we use in our work have improved so dramatically in the last few decades and how this is mostly down to the frequently disparaged study of microbes. While everyone can get behind studying bacteria that cause life-threatening diseases like typhoid fever and cholera, I think that it is often harder to convince people of the value of studying ordinary and sometimes obscure bacteria that do not directly affect human health. However, over the years, such studies have revolutionized many aspects of our lives. They have done so by adapting the biomolecules identified in these studies to produce tools that are now indispensible, not only for researchers like myself who are trying to understand how genes work and what happens to people when these genes go wrong, but also to epidemiologists, doctors, archeologists, historians, forensic scientists, and farmers. In the process, these tools have made the Biotech industry into a multibillion-dollar operation.
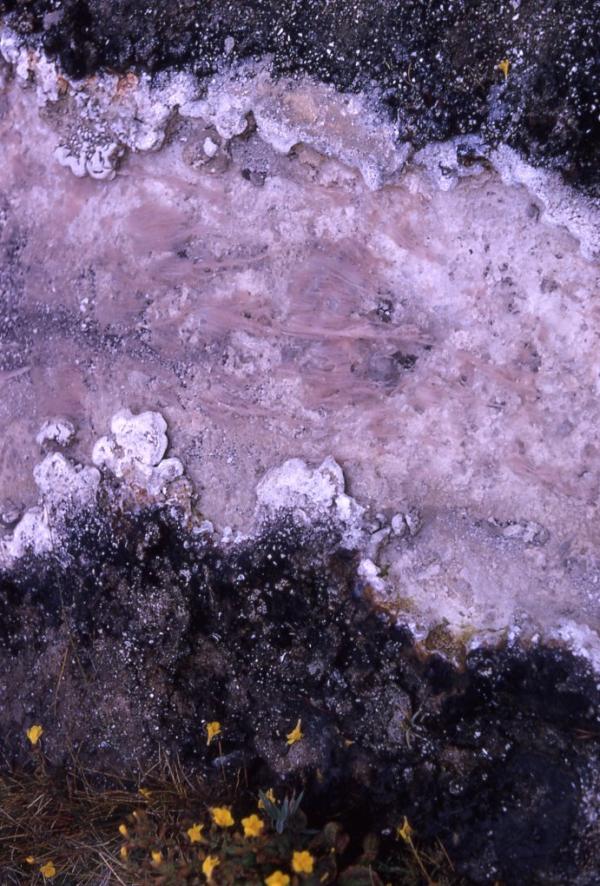
Thermophilic bacteria in Yellowstone National Park; Photographer unknown; 1966
In the 1980s, when I started graduate school, the field of molecular biology was undergoing amazing growth. Three tools that made that growth possible emerged from research on sometimes obscure bacteria: restriction enzymes, which are bacterial proteins able to cut DNA at very specific places; T4 DNA ligase, a protein made from a bacterial virus, that can be used to stick pieces of DNA together; and plasmids, circles of DNA that replicate in bacteria and that can be made to carry a protein “payload.” Together, these tools enabled researchers to make large amounts of bio-identical versions of human proteins, like insulin and human clotting factor VIII. This advancement transformed the treatment of diseases like diabetes and hemophilia, making medications not only less expensive but also safer, by eliminating the risk associated with using human or animal sources of proteins. The new tools also made possible the initial sequencing of the human genome, the development of new and better vaccines, as well as ways of testing for infectious agents like HIV and Ebola. They have made possible our understanding, at the molecular level, of hundreds of disease-relevant proteins that in turn has enabled rational drug design to improve human health.
The study of bacteria living in the thermal springs of Yellowstone National Park by Thomas Brock and Hudson Freeze in the 1960s enabled the development, decades later by Kary Mullis, of the technique known as the Polymerase Chain Reaction (PCR). This technique, for which Mullis was awarded a Nobel Prize in 1993, can make millions of copies of a DNA sequence from vanishingly small amounts of material. PCR revolutionized disease diagnostics, allowing a more rapid and precise identification of infectious agents than ever before. Rapid identification is often critical in containing the spread of infectious diseases, as recently demonstrated by Julie Segre and Tara Palmore here at the NIH. PCR has contributed to our understanding of human history by making it possible to study DNA from 500 year old human remains found in a British parking lot that turned out to be King Richard III of England, and from a tiny finger bone found in a Siberian cave that turned out to belong to an early relative of humans, a Denisovan girl, who lived around 41,000 years ago. It is used by zoos to reduce the deleterious effects of inbreeding and by courts to establish paternity. It has forever changed forensic science, allowing vital clues to be obtained from crime scenes that would have been inconceivable in the pre-PCR age. It has made whole genome sequencing a reality and kick-started the field of personalized medicine. These developments would have been difficult, if not impossible, to imagine, when the initial work on bacteria was first started decades ago.
And just when you thought that there would be no more surprises, the CRISPR/Cas9 system comes along. CRISPR, or clustered regularly interspaced short palindromic repeats, along with the bacterial protein Cas9, form part of a system that protects bacteria from the viruses that infect them. Labs like those of Jennifer Doudna and Emmanuelle Charpentier, with input from NIH’s own Eugene Koonin, have modified the CRISPR/Cas9 system to make it into a relatively precise tool that can be used to fix mutations in the human genome. (Unlike restriction enzymes that make many thousands of cuts in the human genome, CRISPR/Cas9 can be used to cut at a single user-specified location). This technology may one day be used for improving the quality of life for people with certain genetic disorders. For example, in conjunction with our new-found ability to reprogram adult cells and the emerging field of 3-D organ printing (more about both in a later blog post perhaps), it may one day be possible to carry out transplants using organs generated from the patient’s own cells after CRISPR/Cas9-mediated repair. The beauty of this approach is that it would not require the subsequent life-long immune suppression necessary with allogeneic transplants. While this approach is not quite ready for prime time, many labs, including my own, are putting the CRISPR/Cas9 system to work to study patient cells and animal models of human diseases, because it allows key questions about the mechanisms of disease pathology to be addressed in a much more rapid and specific way than previously possible. And for that we owe a big shout out to all those bacteriologists out there that have made this sort of technology possible.
Related Blog Posts
This page was last updated on Monday, January 29, 2024