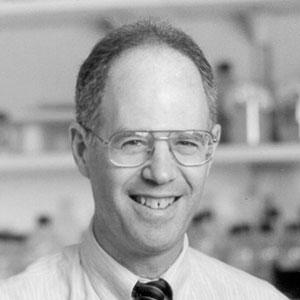
Reed Brendon Wickner, M.D.
NIH Distinguished Investigator
Genetics of Simple Eukaryotes Section, Laboratory of Biochemistry & Genetics
NIDDK
Research Topics
Our studies of yeast infectious diseases provided the first proof of the prion concept, and allowed extensive characterization of several viral and prion systems, made possible by the ease of genetic studies in this simple eukaryote. This work has already facilitated studies of analogous mammalian systems. We have discovered several anti-prion systems, elucidated the architecture of several yeast prions, shown that prions exist as a 'cloud' of distinct variants that segregate and mutate on growth, and explored the biology and population genetics of yeast prions. We have proposed the only model that has been put forward to explain how prion proteins can template their own conformation, the central mystery of prions.
Current Research
In 1994, I discovered two infectious proteins (prions) of the yeast, Saccharomyces cerevisiae. Genetic evidence led us to propose that the non-Mendelian genetic elements, [URE3] and [PSI], are each prions (Fig. 1). A prion is an altered form of a normal protein that may have lost its normal function, but has acquired the ability to convert the normal form of that protein to the abnormal (prion) form. [URE3] is an altered form of the URE2 protein whose normal function is to turn off utilization of poor nitrogen sources if a good nitrogen source is present. [PSI] is the prion form of the SUP35 protein, which is involved in translation termination. We showed that the prion domain of Ure2p is the N-terminal 65 amino acid residues, a region rich in asparagine, serine, and threonine.
Infectious amyloid
The normal form of Ure2p is a soluble dimer with the prion domain unstructured. The prion domain readily forms amyloid in vitro and, when mixed with the native full-length Ure2p, also induces the latter to form amyloid. We showed that amyloid of recombinant Ure2p is infectious for yeast cells, infecting them with the [URE3] prion. The prion domain is sufficient for infectivity, and is sufficient to propagate the prion in vivo. Cells infected with amyloid of recombinant Ure2p carry any of a large number of heritable prion variants. Extracts of a variant are again infectious for yeast and transmit only the variant with which they are infected. Infectious particles are larger than 40 mers.
Architecture of yeast prions
We found that the amyloid of Ure2p consists of a beta-sheet core region (Ure2 residues 1-65) (Fig. 2), a C-terminal domain similar in structure to glutathione-S-transferases (Ure2 residues 95-354), and a flexible tether connecting these two regions (Ure2 residues 66-94). In Ure2 amyloid, monomers are connected by interactions between the N-terminal domains. We found that randomly shuffling the prion domain of Ure2p or of Sup35p and integrating the shuffled prion domain into the chromosome in place of the wild-type sequence produces proteins, all of which can still be prions. This means that amino acid content (and not sequence) is the prime determinant of prion-forming ability. It also argues that the prion domain assumes a parallel in-register beta sheet structure, not anti-parallel beta sheet or beta helix. In collaboration with Rob Tycko of the NIDDK, we have confirmed this prediction for the prion domains of Ure2p and Sup35p (and that of Rnq1p determining the [PIN] prion), and found the same result for several shuffled prion domains of Ure2p and Sup35p as well (Fig. 3). We also found that two different variants of Sup35NM (different [PSI] variants) also have the in-register parallel beta sheet structure. Electron microscopic (EM) determinations of the mass/length of yeast filaments confirm this picture and rule out some alternatives as well. EM measurements of filament diameters show that the in-register beta sheet is multiply folded along the long axis of the filament.
Parallel in-register architecture of prions explains how a protein can be a gene (Fig. 3)
The most remarkable thing about a prion, particularly a yeast prion, is that a protein can be a gene. A given prion protein can be in any of several different prion forms, with very different biological properties, called prion variants. By what mechanism does the protein transmit its conformation to new native molecules joining the ends of the filaments? We suggested that different prion variants have the beta sheet folds in different locations. It did not escape our notice that the in-register beta sheet architecture can explain inheritance of prion variants. The positive interactions among aligned amino acid side chains force the protein monomer newly joining the end of the filament to assume the same conformation as the other molecules in the fiber, with the turns/folds in the same location. In brief, the same forces that maintain the in-register architecture drive the new molecule joining the end of the filament to have the same conformation as molecules already in the filament. Recently, we have obtained evidence for the location of the folds in the sheet (turns or loops in the molecules) of infectious Sup35p prion domain amyloid.
Chaperone and other effects on prions
We showed that propagation of the [URE3] prion requires the Hsp104 disaggregating chaperone, as well as the Hsp70 family chaperone Ssa2. We found that overproduction of the Hsp40 chaperone Ydj1p cures [URE3], as does the GTP-exchange factor Sse1p. We found that overproduction of the Batten disease-related protein Btn2 or its paralog Cur1 also cures [URE3]. Btn2 cures the [URE3] prion by collecting the Ure2p aggregates at a single site in the cell. Deletion ofBTN2 results in increased [URE3] seed number, showing that normal levels of Btn2p have the same function. In fact, most [URE3] variants that arise in a btn2 cur1 mutant are cured by just replacing the normal levels of both proteins, showing that these proteins normally have anti-prion actions without artificial over production. The [URE3] prion variants that are cured by normal levels of Btn2 and Cur1 are specifically those of low seed number. [URE3] variants with high seed number require overproduction of Btn2 or Cur1 to be cured. Btn2 and Cur1 work together with Hsp42, and Hsp42 overproduction also cures [URE3] in a process that requires Btn2/Cur1.
Anti-prion systems
Hsp104, when overproduced, cures the [PSI+] prion. With Btn2p and Cur1p as our model, we examined the ability of normal levels of Hsp104 to cure [PSI+] variants. To do this we used the hsp104(T160M) mutant shown by Masison’s lab to be incapable of curing [PSI+] even when overproduced, but able to stably maintain this prion. We found that [PSI+] arises >10 times more frequently in this mutant than in the wild type. Moreover, more that half of [PSI+] variants arising in the hsp104(T160M) strain are cured when the normal level of the normal Hsp104 is restored. Thus, Hsp104 is acting normally as an anti-prion system culling a large fraction of the [PSI+] variants as they arise.
We then devised a general screen for anti-[PSI+] cellular components, and found that about half of [PSI+] variants arising in an siw14Δ mutant are cured on restoration of the normal levels of Siw14p. The Rolfes lab has shown that Siw14p is a pyrophosphatase specific for 5-pyrophospho-inositol pentakisphosphate (5PP-IP5 pyrophosphatase). The soluble inositol poly/pyro phosphates are multi-functional molecules derived from phosphatidyl inositol phosphates. Our detailed study showed that nearly all [PSI+] variants require inositol poly/pyro-phosphates for their propagation, and that any of three species, IP6, 5PP-IP4 or 5PP-IP5, can support this prion. We also showed that, in the absence of the 5PP-IPs, 1PP-IP5 is an inhibitor of [PSI+] propagation. Current work is exploring the mechanism of these effects.
Our screen for anti-[PSI+] systems showed that the Upf proteins, involved in mRNA nonsense-mediated decay, also comprise an anti-prion system. Normally, the Upf proteins (Upf1, Upf2 and Upf3) form a complex with eachother and with Sup35p. Our evidence indicates that it is these interactions with each other and with Sup35p that directly block prion formation, and can cure most of the [PSI+] prions formed in the absence of any one of the Upf proteins. Upf1p binds to the Sup35p amyloid filaments in [PSI+] strains, and inhibits amyloid formation in vitro by purified Sup35p (but not by Ure2p). We suggest that the Upf proteins either compete with the filaments for free Sup35p monomer preventing filament elongation, or block filament elongation by binding to the ends of the filaments.
In each of these cases – Btn2p, Cur1p, Hsp104, Siw14 and Upfs – the normal level of the protein can cure a large fraction of the prion variants formed in the absence of the protein (or an activity of the protein). This indicates that there is a great deal more prion formation going on in normal cells than is generally apparent, but that these systems cure the large majority of prions as they arise.
We used a transposon mutagenesis method to search for genes protecting yeast from the toxic effects of prions. We found that mutation of LUG1/YRL352W was without substantial effect in [ure-o] cells, but resulted in a dramatic growth defect in cells carrying a [URE3] prion variant that has little effect on normal cells. Lug1p is known to be an F-box protein with a substrate-specifying role for a class of E3 ubiquitin ligases, but its substrate is not known. We found that the growth defect of lug1Δ [URE3] strains is suppressed by overproduction of Hap4p or a gln1 mutation. Mutations in a wide array of chaperone genes was also strongly selected against in the [URE3] strain compared to the [ure-o] strain. Thus, the cell both blocks prion formation, and has other means of preventing the pathologic effects of those prion variants that manage to get through the other systems.
Biological roles of yeast prions
We have shown that both [URE3] and [PSI] are diseases of yeast that, in spite of their infectious nature and ability to arise de novo rather often, are not found in a survey of wild strains. The partial conservation of sequence of the Ure2p prion domain has been interpreted as evidence that prion formation ability is conserved. We showed that the Candida glabrataUre2p has an N-terminal domain that is quite similar to the prion domain of the S. cerevisiae Ure2p, but cannot form a prion. In contrast, the N-terminal domain of the C. albicans Ure2p is much more diverged from the cerevisiae Ure2p, but can form a prion. We further found that the Ure2p prion domain is important to stabilize the full-length protein against degradation in vivo. Thus, this prion domain has a function independent of prion formation. The Ure2p of S. castellii is incapable of becoming a prion, thus confirming that its raison d'etre is not prion formation.
We showed that among variants of [PSI] and [URE3] are those that are lethal or near-lethal to the cell, recovered by genetic tricks that prevent the lethality. These lethal variants are very common among total isolates. This finding confirms that prion-forming ability of Sup35p and Ure2p are not adaptive. Yeast prions are molecular degenerative diseases, and their study will continue to shed light on the processes leading to human amyloid diseases.
We have extensively examined prion variants of [URE3] derived from the Ure2ps of various Saccharomyces species. We find that the rapid variation of Ure2 prion domain sequence has resulted in a species barrier for prion transmission between many pairs of Ure2ps. However, the degree of species barrier depends on which prion variant is being tested. Moreover, passage of a prion variant through another species can leave its variant characteristics intact. Perhaps even more striking is the Sup35p of wild strains of S. cerevisiae. There are three groups of sequence polymorphs common in wild strains, and we found that transmission of the [PSI] prion from one to another is largely blocked. We propose that these polymorphs of Sup35p have been selected by their ability to block [PSI] propagation, much as the human polymorphism at PrP residue 129 blocks propagation of spongiform encephalopathies and is proposed to have been selected by this ability.
We have recently estimated the frequency of outcross mating of S. cerevisiae using two approaches and used this estimate and the known rarity of the yeast prions to determine the minimum detriment to yeast of carrying one of these prions. Of course, these are minimum estimates of detriment because only the mildest yeast prion variants are compatible with survival in the wild. The frankly lethal variants of [PSI] and [URE3] would quickly doom a wild strain. As part of this study, we found that some alleles of RNQ1 encode a truncated Rnq1p that is unable to propagate the [PIN] prion.
An enzyme-based prion
We have also described a different kind of prion, an enzyme whose active form is necessary for the activation of its own inactive precursor. This prion is simply the vacuolar protease B of yeast. It is not novel to find a self-activating protease, but to find a protease acting as a gene is unique. Moreover, many self-modifying proteins are known and some may prove to be this type of prion as well.
Non-prion amyloids
The Pmel17 protein of melanocytes is necessary for proper melanin biogenesis in mammalians, and this protein is known to form filaments in melanosomes that are thought to be amyloid. We found that only the repeat domain of Pmel17 forms amyloid, and only at the mildly acid pH typical of the lysosome-related melanosome. Pmel17 of other vertebrates also have a repeat domain, but with completely different repeats. We showed that these repeat domains also form amyloid in vitro, with the same specificity for mildly acid pH. We have also confirmed the amyloid nature of the curli proteins csgA and csgB of E. coli, and have obtained evidence that csgA is not an in-register parallel structure. We have recently shown that amyloid of transthyretin is an exception to the rule that pathological amyloids of humans are in-register parallel structures.
Double-stranded RNA viruses of yeast
Our lab has developed the classical genetics, molecular cloning, and enzymology of the replication of the L-A virus, its satellites, and their interactions with the host. We have established template-dependent in vitro replication and transcription systems for this virus, the first for a dsRNA virus, and have used them to elucidate the sites on the RNA necessary and the viral proteins that participate in these processes. We have defined the packaging site on the RNA, the packaging domain on the viral Pol protein, and obtained evidence for the mechanism of the packaging process.
The L-A virus has two open reading frames, the 5' gag, encoding the major coat protein, called Gag, and the 3' pol, encoding a multifunctional protein domain called Pol that includes the RNA-dependent RNA polymerase. Pol is expressed only as a Gag-Pol fusion protein formed by a -1 ribosomal frameshift event whose mechanism is identical to that used by retroviruses for the same purpose. We have described chromosomal genes whose mutation alters the efficiency of ribosomal frameshifting and thereby impairs virus propagation. We discovered a set of chromosomal genes, SKI1, SKI2, SKI3, SKI6, SKI7, and SKI8, that repress viral propagation and prevent the L-A virus and its satellite RNAs from harming the cell. Our data indicate that SKI2, SKI3, SKI6, SKI7, and SKI8 control viral propagation by inhibiting the expression of the viral messages because they lack 3'poly(A).
Applying our Research
It has proven far easier to check if human systems are the same as their homologs and analogs in yeast or in other model organisms than it has to discover how the various systems work de novo using human systems and cells. Our work studying yeast viruses and prions has demonstrated this for these infectious diseases. Specifically, our yeast anti-prion systems may have homologs or analogs in human cells that can be used to treat amyloid diseases in the same way that the humoral, cellular and innate immune systems are manipulated to counter viral and bacterial infections. The yeast Ski proteins act as an anti-viral system, and have clear homologs in humans and other mammals. We suspect that these human homologs may be manipulated for the treatment of some human viral infections.
Need for Further Study
Further studies in our group will examine (1) the more detailed structure of the yeast prion amyloids of Ure2p, Sup35p, and Rnq1p, and other amyloids; (2) the mechanisms of the anti-prion systems we have discovered; and (3) genetic screens to find other proteins affecting prion generation and propagation, including more anti-prion components. We are also further exploring the biology and pathology of known yeast prions.
Biography
- Elected Fellow, American Association for Advancement of Science, 2003
- Elected National Academy of Sciences, 2000
- Elected American Academy of Microbiology
- Elected American Academy of Arts and Sciences, 1998
- American Society of Clinical Investigation, 1985
- Medical Internship, North Carolina Memorial Hosp., 1967
- M.D., Georgetown University, 1966
- B.A. Cornell University, 1962 (mathematics)
Selected Publications
- Wu S, Edskes HK, Wickner RB. Human proteins curing yeast prions. Proc Natl Acad Sci U S A. 2023;120(45):e2314781120.
- Son M, Wickner RB. Antiprion systems in yeast cooperate to cure or prevent the generation of nearly all [PSI(+)] and [URE3] prions. Proc Natl Acad Sci U S A. 2022;119(28):e2205500119.
- Son M, Wickner RB. Nonsense-mediated mRNA decay factors cure most [PSI+] prion variants. Proc Natl Acad Sci U S A. 2018;115(6):E1184-E1193.
- Edskes HK, Mukhamedova M, Edskes BK, Wickner RB. Hermes Transposon Mutagenesis Shows [URE3] Prion Pathology Prevented by a Ubiquitin-Targeting Protein: Evidence for Carbon/Nitrogen Assimilation Cross Talk and a Second Function for Ure2p in Saccharomyces cerevisiae. Genetics. 2018;209(3):789-800.
- Gorkovskiy A, Reidy M, Masison DC, Wickner RB. Hsp104 disaggregase at normal levels cures many [PSI(+)] prion variants in a process promoted by Sti1p, Hsp90, and Sis1p. Proc Natl Acad Sci U S A. 2017;114(21):E4193-E4202.
Related Scientific Focus Areas
Microbiology and Infectious Diseases
View additional Principal Investigators in Microbiology and Infectious Diseases
Molecular Biology and Biochemistry
View additional Principal Investigators in Molecular Biology and Biochemistry
This page was last updated on Monday, April 21, 2025